Synthetic Biology Tools for Engineering Microbial Cells to Fight Superbugs
- 1Facultad de Ciencias Químicas, Universidad Autónoma de Nuevo León (UANL), San Nicolás de los Garza, Mexico
- 2Centro de Investigación en Biotecnología y Nanotecnología, Facultad de Ciencias Químicas, Parque de Investigación e Innovación Tecnológica, Universidad Autónoma de Nuevo León, Apodaca, Mexico
With the increase in clinical cases of bacterial infections with multiple antibiotic resistance, the world has entered a health crisis. Overuse, inappropriate prescribing, and lack of innovation of antibiotics have contributed to the surge of microorganisms that can overcome traditional antimicrobial treatments. In 2017, the World Health Organization published a list of pathogenic bacteria, including Enterococcus faecium, Staphylococcus aureus, Klebsiella pneumoniae, Acinetobacter baumannii, Pseudomonas aeruginosa, and Escherichia coli (ESKAPE). These bacteria can adapt to multiple antibiotics and transfer their resistance to other organisms; therefore, studies to find new therapeutic strategies are needed. One of these strategies is synthetic biology geared toward developing new antimicrobial therapies. Synthetic biology is founded on a solid and well-established theoretical framework that provides tools for conceptualizing, designing, and constructing synthetic biological systems. Recent developments in synthetic biology provide tools for engineering synthetic control systems in microbial cells. Applying protein engineering, DNA synthesis, and in silico design allows building metabolic pathways and biological circuits to control cellular behavior. Thus, synthetic biology advances have permitted the construction of communication systems between microorganisms where exogenous molecules can control specific population behaviors, induce intracellular signaling, and establish co-dependent networks of microorganisms.
Introduction
With the increase in clinical cases of bacterial infections with multiple antibiotic resistance, the world has entered a health crisis. Overuse, inappropriate prescribing, and lack of innovation of antibiotics have contributed to the surge of microorganisms that can overcome traditional antimicrobial treatments (Ventola, 2015). In 2017, the World Health Organization published a list of pathogenic bacteria, including Enterococcus faecium (E. faecium), Staphylococcus aureus (S. aureus), Klebsiella pneumoniae (K. pneumoniae), Acinetobacter baumannii (A. baumannii), Pseudomonas aeruginosa (P. aeruginosa), and Escherichia coli (E. coli) (ESKAPE) (World Health Organization, 2017). These bacteria have the ability to adapt to multiple antibiotics and transfer their resistance to other organisms; therefore, studies to find new therapeutic strategies are needed. One of these strategies is synthetic biology geared toward developing new antimicrobial therapies.
Synthetic biology is founded on a solid and well-established theoretical framework that provides tools for conceptualizing, designing, and constructing synthetic biological systems (Perrino et al., 2021). Recent developments in synthetic biology provide tools for engineering synthetic control systems in microbial cells. Applying protein engineering, DNA synthesis, and in silico design allows building metabolic pathways and biological circuits to control cellular behavior (McCarty and Ledesma-Amaro, 2019). Thus, synthetic biology advances have permitted the construction of communication systems between organisms where exogenous molecules can control specific population behaviors, induce intracellular signaling, and establish co-dependent networks of microorganisms (Hennig et al., 2015; McCarty and Ledesma-Amaro, 2019).
The applications of synthetic biology systems (artificial circuits and functions within biological systems) include producing biologically based products in agriculture, industry, environmental, and healthcare studies. In this review, we highlight recent works of the impact of synthetic biology on genetic engineering to modify antibiotics and enhance antibiotic production, engineered phages, microbial control systems as an alternative to fight antibiotic resistance, and some other synthetic biology tools to engineer microbial communities (Figure 1).
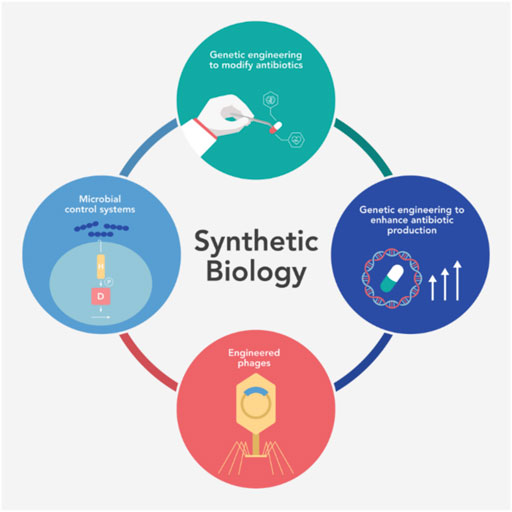
FIGURE 1. Recent works have demonstrated the impact of synthetic biology and the tools and techniques that the field has developed to allow genetically modifying antibiotics, enhancing antibiotic production, developing engineered phages, and designing microbial control systems.
Genetic Engineering to Modify Antibiotics
Organisms are naturally capable of producing metabolites with antimicrobial characteristics. Unfortunately, “wild-type” antibiotic producers have poor production and low titers; however, with the unravel of novel biosynthesis mechanisms of that produce antibiotics and the tools provided by synthetic biology, it is possible to engineer the capacities of organisms to over-produce and diversify these metabolites (Breitling and Takano, 2015; El Karoui et al., 2019; Zhang et al., 2020).
One of the most interesting antibiotic synthesis mechanism is the multimodal enzymatic complex presented in biosynthetic cluster genes (BCG). It is composed of non-ribosomal peptide synthetases (NRPS), polyketide synthases (PKS), and combinations. NRPS and PKS are multimodal enzymatic complexes that are responsible for assembling non-ribosomal peptides (NRP) and polyketides, respectively, and giving them an active chemical structure (Martínez-Núñez and López, 2016; Nivina et al., 2019; Hwang et al., 2020). With the tools provided by synthetic biology and applying some engineering to these enzyme complexes, it is possible to attack the problem of low production and limited diversity of antibiotics.
As a first approach to tackle low production and diversity, antimicrobial compounds members of the NRP group have been addressed especially the family of antibiotics known as glycopeptides (Yim et al., 2014). Some authors had focused their efforts on diversifying these antibiotics, such as Yim et al., who used combinations of 13 scaffold-modifying enzymes from 7 GPA BCGs to be introduced into Streptomyces coelicolor (S. coelicolor). Among these combinations, nine new compounds were reported. Interestingly, eight of those compounds had antimicrobial activity against vancomycin-resistant Enterococcus faecalis (E. faecalis), exhibiting a MIC between 0.5–4 μg/ml (Yim et al., 2016). One of the promising newcomers in the glycopeptide family is corbomycin. Despite its outstanding clinical performance, natural production with Streptomyces sp. has poor performance (Culp et al., 2020). Xu et al. used a glycopeptide antibiotic heterologous expression system (GPAHex) to enhance the expression of genes for corbomicyn synthesis in S. coelicolor, obtaining a 19-fold increase in titers using this platform (Xu et al., 2020).
It is also possible to find that combinations between NRPS and PKS modules can give rise to more classes of antibiotics, such as lipopeptides. Daptomycin is a clinically significant lipopeptide antibiotic used primarily against methicillin-resistant Staphylococcus aureus (MRSA), naturally produced in Streptomyces roseosporus (S. roseosporus). Unfortunately, similar to other antibiotic natural producers, “wild-type” S. roseosporus has low daptomycin titers (Ye et al., 2014). Recently, Ji et al., using a “top-down” synthetic biology approach, achieved an increase in total lipopeptide production up to ∼2,300%, where up to 40% was daptomycin (Ji et al., 2022).
Not only do combinations between NRPS and PKS give rise to the production of antibiotics, in some microorganisms, these enzyme complexes are joined by other families of enzymes such as fatty acid synthases (FAS). Initially discovered in Dickeya zeae, the new family of zeamines is one example of naturally occurring antibiotics resulting from the interaction between NRPS, PKS, and FAS (Wu et al., 2010). Zeamines drew the scientific community’s attention for their potent microbicidal activity against Gram-positive and Gram-negative bacteria. Unfortunately, Dickeya zeae has poor production of these antibiotics (Liao et al., 2014). Therefore, different authors, such as Masschelein et al., have studied other natural producers of zeamines, such as Serratia plymuthica (S. plymuthica) RVH1. Using in-frame deletion of biosynthetic genes, some of the mechanisms involved in synthesizing these antibiotics have been uncovered. This has been an outstanding contribution to synthetic biology for future combinatorial biosynthesis and bioengineering to produce new antibacterial compounds (Masschelein et al., 2013; Masschelein et al., 2015).
In addition to the approaches made by the combination of enzyme complexes, metabolic engineering offers another perspective to improve and diversify the production of antibiotics. Metabolic engineering aims to understand the networks of cellular metabolism and redesign them to improve productive capacities (Olano et al., 2008; Kim et al., 2016; Palazzotto et al., 2019). The efforts provided by metabolic engineering usually focus on redirecting metabolic fluxes, regulating BCGs and enzymes causing bottlenecks.
Discovering the relationships between intermediate metabolites and the biosynthesis of antibiotics have allowed the scientific community to improve yields in antibiotic production. An example of this is the regulation of the metabolite S-Adenosylmethionine (SAM). Although SAM is an essential precursor in methylation processes, this compound is involved in antibiotic biosynthesis in different species (Huh et al., 2004; Wang et al., 2007). Authors, such as Cai et al., manage to increase the yields in bacitracin production in Bacillus licheniformis (B. licheniformis), analyzing the SAM synthetic pathway. Authors, such as Cai et al., through analyzing the SAM synthetic pathway, have managed to increase the yields in bacitracin production up to 28.97% in B. licheniformis with a combination of different synthetic biology techniques such as heterologous expression, deletion, and overexpression of different genes (Cai et al., 2019; Cai et al., 2020).
Up to this point, the described studies have shown that the elucidation of the different mechanisms involved in the biosynthesis of antibiotics and the tools provided by synthetic biology have allowed the redesign of organisms by engineering them to improve their productive capacities and thus contributing to the fight against antimicrobial resistance. Although there are still some critical challenges for the continuous application of synthetic biology strategies to diversify antibiotics, the next few years promise to be rewarding for discovering new antibiotic compounds.
Genetic Engineering to Enhance Antibiotic Production
Different microorganisms are sources of other compounds used as antimicrobial agents. A variety of genetic material codes those metabolites and accessing this genetic diversity could increase the possibility of finding new or better ways to fight resistant microorganisms. Genetic techniques like gene mutation and the ability to control cellular functions at the genetic level could improve antimicrobial biosynthesis (Miao et al., 2006; Ma et al., 2017). Arafat et al. (2021) observed changes in antibiotic production, exposing Streptomyces graminofaciens (S. graminofaciens) to UV light. Studying the cellular function of various genes have allowed researchers to produce higher amounts and even better antibiotics. Studies of the gene cluster of the nucleoside antibiotic A201A in Marinactinospora thermotolerans (M. thermotolerans) SCSIO 00652 (Zhu et al., 2012) have led to improving its production; this has also been accomplished through genetic modification for the biosynthesis of amphotericin analogs in Streptomyces nodosus (S. nodosus) by disruption of amphDIII and amphL (Byrne et al., 2003). Makitrynskyy developed a manipulation of AdpA regions of Streptomyces ghangensis (S. ghangensis) (Makitrynskyy et al., 2021), giving researchers the understanding of a metabolic pathway to improve a specific antimicrobial biosynthesis. By understanding metabolic pathways and applying different gene engineering techniques, research can improve antimicrobial production through punctual modifications to regulate specific genes, demonstrating that genetic modifications to control biosynthesis pathways could improve antimicrobial production and its activities (Song et al., 2017; Li D. et al., 2021; Li Y.-P. et al., 2021).
Similarly, intended gene modifications can improve antimicrobial production, improve the metabolic flux to precursor availability, and enhance biosynthesis (Meng et al., 2017; Moosmann et al., 2020). These modifications could increase the concentration of antimicrobial precursors, such as (Shomar et al., 2018) expressing the gene cluster for carbapenem in E. coli producing antibiotics with a 60-fold increase. Also, modifying metabolic pathways, like carbon flux from the pentose phosphate pathway (PPP) to glycolysis by modifying zwf1 and zwf2 on Streptomyces lividans (S. lividans) (Butler et al., 2002) have led to increased glycolysis intermediates needed for antibiotic production.
A better bio-factory could be achieved by introducing genes in or from a different host taking advantage of its synthesis route (Han et al., 2012; Sakai et al., 2012; Makitrynskyy et al., 2021). Chen et al. (Chen et al., 2009) studied the expression of a gene cluster of Streptomyces cacaoi (S. cacaoi) in S. lividans TK24. However, polyoxin production by S. lividans TK24 was entirely the polyoxin H derivate due to the lack of genes for hydroxylation/carboxylation, leading to the formation of polyoxin A/F derivates. Eustáquio et al. (Eustáquio et al., 2004) expressed the gene cluster to synthesize novobiocin in S. coelicolor. Two S. coelicolor mutants were obtained by modifying the novO gene, an 8′-unsubstituted novobiocin by inactivation of novO, and a chlorine atom at C-8′ by expressing the clo-hal gene from the clorobiocin gene cluster. Wang et al. (Wang et al., 2021) expressed CYP genes of the mushroom Ganoderma lucidum in Saccharomyces cerevisiae (S. cerevisiae) to produce a derivate of 3,28-dihydroxy-lanosta-8,24-dien-26-oic acid. By improving the copy number of two resistance plasmids, the production of this new antibiotic was increased 8.2 folds compared to the control strain. The expression of non-ribosomal peptide synthetases in Bacillus subtilis (B. subtilis) was studied by Eppelmann et al. (Eppelmann et al., 2001) by introducing the bacitracin biosynthesis gene cluster of B. licheniformis. B. subtilis showed comparable self-resistance to bacitracin due to the gene replacement and increased bacitracin A production due to the high-level expression of the bacitracin synthetase and the higher growth rate compared to B. licheniformis. Similarly, Wu et al. (Wu et al., 2015) expressed the gene ram29 of the ramoplanin producer Actinoplanes sp. into Streptomyces fungicidius (S. fungicidius)to produce monomannosylated enduracin derivates.
The main goal of creating an engineered microorganism is the increased production of a specific antimicrobial. Nevertheless, when genetic engineering is focused on manipulating foreign genes in an organism, various reasons could affect its biosynthesis. Modifying genes of a particular biosynthesis or metabolic pathway may be enhanced in production but could decrease its antimicrobial activity. Even in the same genre, the differences between strains give the possibility of obtaining derivates of the intended antimicrobial, which could also modify its action. Thus, knowing how a microorganism’s genetic material helps it survive in the microbiome can help us acquire the tools to manipulate it and use it to our advantage, increasing the possibility of obtaining new and better antibiotics.
Engineered Phages to Fight Superbugs
Phages are viruses that infect specific bacteria and are the most abundant organisms on Earth (up to 2.5 × 108 phages per mL in water) (Bergh et al., 1989). Phages need bacteria to replicate and survive; thus, naturally, they work as bacteria controllers. Phages were discovered at the beginning of the XX century by Frederick Tort and Felix d’Herelle (Sharma et al., 2017), but antibiotics rapidly replaced phages in treating microorganism infections. However, due to the emergence of multi-antibiotic resistant bacterial pathogens, phages are considered an alternative way to treat multi-resistant bacteria.
Nowadays, researchers are studying single phages, discovering new phages, and designing phage cocktails as therapies to treat multi-resistant bacterial infections. Natural phages could be enough to fight superbugs, just like the works reporting on the administration of phage cocktails to patients infected by a multi-drug resistant A. baumannii strain, with results showing complete recovery after phage therapy (Schooley et al., 2017). Nevertheless, synthetic biology can improve or extend phage’s abilities to generate variants with unique properties (Yacoby et al., 2007; Edgar et al., 2012). One of the strategies is to design and build phages with a broad host range (Lin et al., 2012). Phages provide a highly specific target of bacterial strains; thus, cocktails are required to fight infections and reduce the possibility that bacterium acquires resistance to any phage. Yehl et al. (2019) have developed a high-throughput strategy to engineer host-range-determining regions (HRDRs) in T3 phage by site-directed mutagenesis. Inspired by antibody specify engineering, this approach reduces disruptions in tail structure, and they call it “phage bodies”.
Following this strategy could reduce the number of phages in cocktails. Using the monophages approach reduces the preparation and purification efforts and there is less potential for complications derived from using phages cocktails, such as phage purification, phage compatibility, making it easier to use as treatment. In addition, cocktails could make bacteria develop “broad-spectrum” mechanisms of phage resistance, such as capsules that avoid phage binding (Schooley et al., 2017).
Furthermore, Ando et al. (2015) swapped tail fiber genes to allow a genetically E. coli to target Klebsiella and vice versa. The authors also demonstrated that synthetic phage cocktails with the same scaffold, but different tails selectively remove bacteria from multi-specie communities. Bacterial biofilms are difficult to eradicate since the physical properties of matrix, the physicochemical properties of the exopolysaccharides, and the heterogeneity of the bacterial cells within the biofilm confers them a strong resistance against chemicals (antibiotics, immunological approaches, and phages). Nevertheless, phages genetically engineered could be used to overcome this problem. Chen et al. (2021) constructed an engineered T4 called T4 Rn11 that exhibits antibiofilm activity against Streptococcus mutants (S. mutants). A reduction in biofilm biomass and formation of microcolonies was achieved using this phage. In a similar work, Pei and Lamas-Samanamud (2014) constructed a T7 phage that produces a lactonase enzyme with broad-range activity for quenching quorum sensing. The modified phage effectively degrades acyl-homoserine lactones (AHLs) from many bacteria. In addition, it inhibited P. aeruginosa and E. coli biofilm formation.
Reducing biofilm is crucial in the treatment of infection caused by microorganisms in cystic fibrosis disease (Dedrick et al., 2019) or in wound infections, but also it is useful in reducing biofilm in medical devices such as catheters (Fu et al., 2010). These devices are responsible for substantial morbidity and mortality among patients.
As we described above, combining phage’s natural abilities with synthetic biology tools can improve the potential for phages by expanding their range of infection and mediating bacterial signaling or expressing enzymes (Bikard et al., 2014) that help eliminate multi-resistant bacteria.
Microbial Control Systems
In the last decade, synthetic biology tools have allowed the construction of microbial control systems by engineering whole living cells to act as biosensors and detect and respond to internal and external signals [quorum sensing (QS)] secreted by pathogens (Benítez-Chao et al., 2021; Perrino et al., 2021). Some QS-based phenotypical behaviors in bacteria are sporulation formation, virulence factors related to invasion, bioluminescence, and population control (Benítez-Chao et al., 2021).
In numerous studies, synthetic genetic circuits have been developed to analyze biological systems and guide their design based on QS. A group of researchers genetically modified E. coli to detect wild-type P. aeruginosa (PAO1), specifically via its QS molecule. Their results demonstrated that engineered E. coli sentinels successfully inhibit PAO1 growth by secreting a novel pathogen-specific engineered chimeric bacteriocin (Gupta et al., 2013). Later, a Lactococcus lactis (L. lactis) to detect E. faecalis was designed in another study. L. lactis was able to produce and secrete peptides that inhibit enterococcal growth and reduce the viability of enterococci in the surrounding area of L. lactis. This engineered system was demonstrated to be effective against multidrug-resistant E. faecium strains (Borrero et al., 2015). Holowko et al. (2016) designed and created a synthetic genetic sensing system using nonpathogenic E. coli as the host based on CRISPRi technology. They moved proteins used by Vibrio cholerae (V. cholerae) for QS into E. coli and showed high sensitivity to the presence of V. cholerae supernatant with tight control of expression of output GFP protein (Holowko et al., 2016). Lubkowicz et al. (2018) developed a probiotic lactic acid bacteria (Lactobacillus reuteri) engineered to detect autoinducer peptide-I (AIP-I), a quorum-sensing molecule produced by Staphylococcus sp. during pathogenesis. Their results showed that the engineered biosensor could detect AIP-I levels under various strenuous conditions in the S. aureus (Lubkowicz et al., 2018). Recently, Wu et al. created a novel whole-cell biosensor to detect bacterial pathogens (P. aeruginosa and Burkholderia pseudomallei) by responding to the relevant QS signal molecules. Results showed that engineered whole-cell biosensors provide rapid and cost-effective detection of waterborne pathogens (Wu et al., 2021).
As described above, quorum sensing is a process in which bacteria communicate with their own species or across species to coordinate cellular behavior. In the last decade, synthetic biology has used the properties of quorum sensing as a tool to build and develop genetic circuits for population control. Thus, scientists seek to design biological systems with predictable behavior. Table 1 displays a summary of the synthetic biology tools to fight antibiotic resistance using genetic engineering to modify antibiotics and enhance antibiotic production, engineered phages, and microbial control systems.
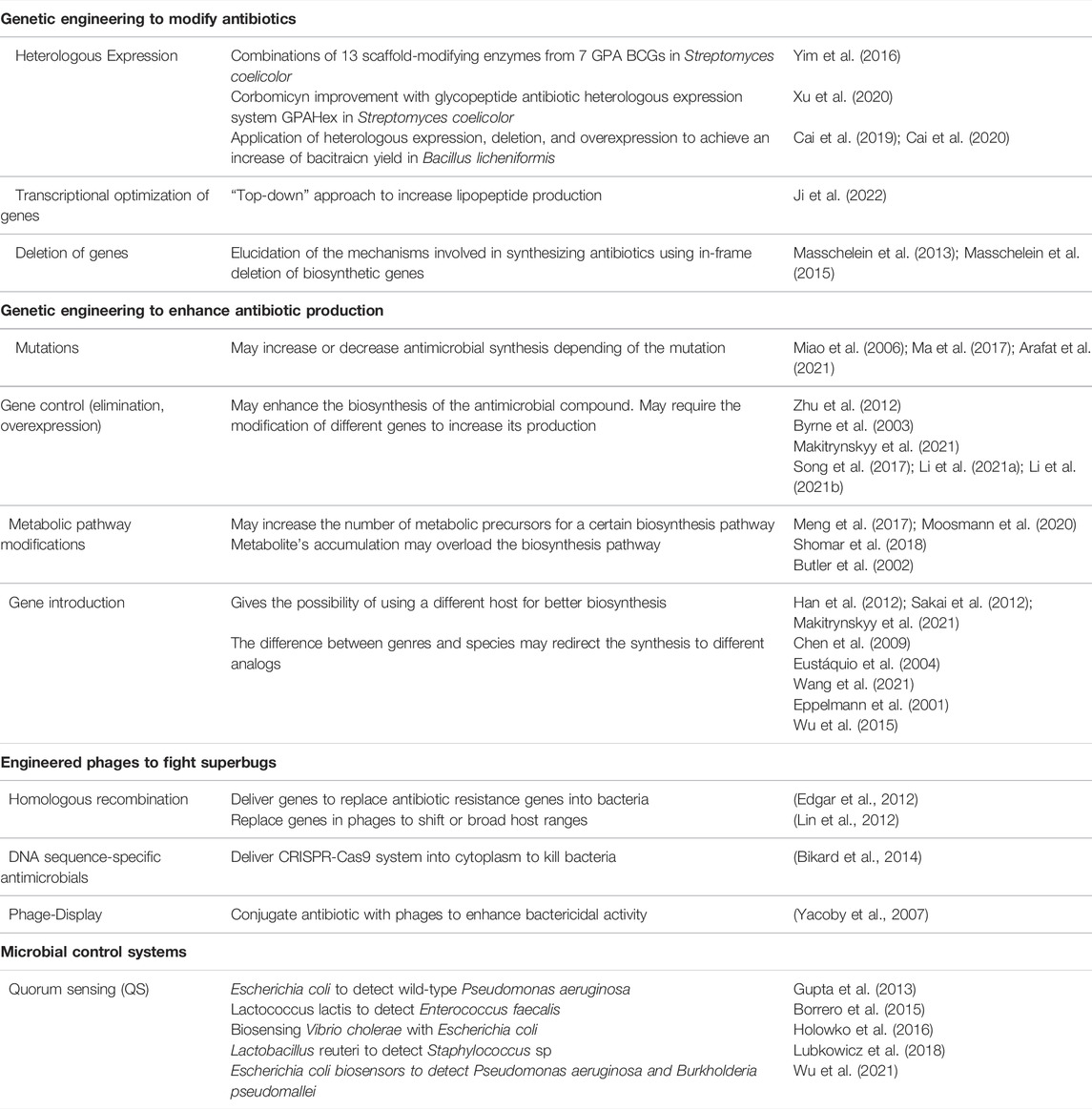
TABLE 1. Synthetic biology tools to fight antibiotic resistance using genetic engineering to modify antibiotics and enhance antibiotic production, engineered phages, and microbial control systems.
Other Synthetic Biology Tools to Engineer Microbial Communities
Synthetic biology uses engineering principles in biological systems in a predictable, controllable, and standardized manner to get new biological insights and create cells with improved abilities. The potential uses of emerging technologies such as the Design-Build-Test-Learn (DBTL) cycle, protein engineering against multidrug-resistant bacteria, and logic gates are described below.
Design-Build-Test-Learn Cycle
As we previously described, synthetic biology is a recently emerging discipline used to design or redesign biological systems and give them improved or new qualities. One of the SynBio engineering principles used to create new biological systems is the Design-Build-Test-Learn (DBTL) cycle (Whitford et al., 2021). DBTL cycle is an increasingly adopted metabolic engineering framework that helps systematize cellular activities and increase their efficacy and generalizability (Opgenorth et al., 2019). Thus, the design of biological circuits and high-throughput screening (HTS) technologies have begun to speed up modern drug discovery cycles and produce new medicines. Each phase of the DBTL cycle is a fundamental component of the cycle. The Design component identifies the problem and selects the desired pathway and host. The Build component selects, synthesizes, and assembles parts for the incorporation into the host. The Test component validates the engineered constructs and strains for target molecule production, transcripts, proteins, and metabolites. This phase generates a significant amount of data. The Learn component analyzes the Test data, and the learnings are used to create a novel testable hypothesis and incorporate them into the next cycle (Petzold et al., 2015; Carbonell et al., 2018). With the DBTL cycle, researchers can rapidly construct new biological systems. Coupling the DBTL cycle with engineering technologies can deliver solutions in drug discovery and solve global problems such as antimicrobial resistance.
Protein Engineering Against Multidrug-Resistant Bacteria
Like endolysins (encoded by bacterial viruses), proteins act by disrupting the bacterial cell wall or other proteins that inhibit the expression of genes related to antibiotic resistance (gene regulators) and leading to cell death. Some proteins alter microbial behavior, the bacterial cell wall and interrupt signals and genes confering multi-drug resistance. Based on these kinds of proteins, synthetic biology techniques are helpful for engineering proteins into making them more stable or efficient. Moreover, synthetic proteins can be created and manufactured such is the case of the Briers et al. (2014) group that engineered endolysins to act as artilysins (outer membrane-penetrating endolysins Briers et al., 2014). One drawback is that endolysins do not have activity against Gram-negative bacteria because of the impermeable lipopolysaccharide layer surrounding their cell wall. Still, artilysins are highly bactericidal against Gram-negative pathogens, including P. aeruginosa and A. baumannii.
Another example is dCas9 which is a “dead” catalytical protein. The system is the same used in CRISPR-Cas9 technology, with the difference that dCas9 can bind to the DNA but not cut the strands. In this way, dCas9 is targeted to suppress gene transcription. Wang and Nicholaou (2017) designed two CRISPR-dCas9 systems to target the mecA promoter in MRSA to repress the gene’s transcription (Wang and Nicholaou, 2017). Although these alternatives are not applied to clinical, they are promising approaches to use alone or combined with antibiotics or another technology.
Logic Gates
An interesting line of research in synthetic biology is that of making microorganisms work as control elements, sending and receiving signals through logic gates. Logic gates are widely used in electronic devices. These devices have electronic circuits that transform an input signal into another output signal or convert two input signals into one output signal (Wang et al., 2011). In synthetic biology, living cells act as control elements; therefore, living cells can be programmed to produce the precisely desired behavior in response to intra or extracellular signals (Morris et al., 2010). Gene regulatory network that cells use to interact and respond to the environmental signals, can be used to program logic circuits to link cellular sensors and actuators. Genetic logic gates rely on direct control of the transcription, activating a promoter. One example is using these genetic elements to program a cell to recognize quorum-sensing signals. Benítez-Chao et al. (2021) designed a whole-cell biosensor to sense and kill MRSA. Using genetic logic gates, they design a genetic circuit where E. coli can recognize quorum-sensing molecules from MRSA and triggers the expression of a bacteriocin that kills MRSA.
Genetic circuits can also be designed to disrupt a metabolic pathway in multi-drug resistant bacteria or to create whole-cell biosensors, responding to a molecule produced by pathogen organisms and producing an antimicrobial compound. Whole-cell biosensors are still in research but have a promising future in controlling pathogen bacteria.
Conclusion
This review presents recent approaches based on synthetic biology as an emerging tool to produce new therapeutic compounds. Synthetic biology provides a wide variety of techniques that aid in the design and construction of microorganisms capable of creating, producing, and enhancing biological functions to use them to tackle antibiotic resistance. Still, there are significant challenges to overcome, like 1) many biological components lack clear description, 2) the construction of biological systems is complicated and can be unpredictable, and 3) bio-circuit test is complicated and time-consuming. Therefore, it is necessary to keep studying and innovating processes before being translated into human medicine.
Author Contributions
Conceptualization, AL-B and JM-R. Writing-original draft preparation AL-B, FB-C, CG-C, JG-C, and JM-R. Writing-review and editing, AL-B, FB-C, CG-C, JG-C, and JM-R. Supervision, AL-B and JM-R. All authors contributed to the article and approved the submitted version.
Funding
We would like to acknowledge Paicyt 2019–2020 and 2020–2021 Science Grant from the Universidad Autónoma de Nuevo León; CONACyT Grants for Basic Science grant 221332; Fronteras de la Ciencia grant 1502, Infraestructura Grant 279957 and Apoyos a la Ciencia de Frontera Grant 316869.
Conflict of Interest
The authors declare that the research was conducted in the absence of any commercial or financial relationships that could be construed as a potential conflict of interest.
Publisher’s Note
All claims expressed in this article are solely those of the authors and do not necessarily represent those of their affiliated organizations, or those of the publisher, the editors and the reviewers. Any product that may be evaluated in this article, or claim that may be made by its manufacturer, is not guaranteed or endorsed by the publisher.
Acknowledgments
CG-C would like to thank the support from Beca Nacional de Maestría 2020–2022 (Scholarship number 1080929).
References
Ando, H., Lemire, S., Pires, D. P., and Lu, T. K. (2015). Engineering Modular Viral Scaffolds for Targeted Bacterial Population Editing. Cell Syst. 1, 187–196. doi:10.1016/j.cels.2015.08.013
Arafat, H. H., Abu-Tahon, M. A., and Isaac, G. S. (2021). Production of Antibiotic Carbomycin from Streptomyces Graminofaciens with High Lipid Content Mutation. Arch. Microbiol. 203, 901–911. doi:10.1007/s00203-020-02085-6
Benítez‐Chao, D. F., Balderas‐Cisneros, F. d. J., León‐Buitimea, A., and Morones‐Ramírez, J. R. (2021). Design and In Silico Analysis of a Whole‐cell Biosensor Able to Kill Methicillin‐resistant Staphylococcus aureus. Biotech App Biochem. 2021, 2210. doi:10.1002/bab.2210
Bergh, Ø., BØrsheim, K. Y., Bratbak, G., and Heldal, M. (1989). High Abundance of Viruses Found in Aquatic Environments. Nature 340, 467–468. doi:10.1038/340467a0
Bikard, D., Euler, C. W., Jiang, W., Nussenzweig, P. M., Goldberg, G. W., Duportet, X., et al. (2014). Exploiting CRISPR-Cas Nucleases to Produce Sequence-Specific Antimicrobials. Nature Biotechnol. 32, 1146–1150. doi:10.1038/nbt.3043
Borrero, J., Chen, Y., Dunny, G. M., and Kaznessis, Y. N. (2015). Modified Lactic Acid Bacteria Detect and Inhibit Multiresistant Enterococci. ACS Synth. Biol. 4, 299–306. doi:10.1021/sb500090b
Breitling, R., and Takano, E. (2015). Synthetic Biology Advances for Pharmaceutical Production. Curr. Opin. Biotechnol. 35, 46–51. doi:10.1016/j.copbio.2015.02.004
Briers, Y., Walmagh, M., van Puyenbroeck, V., Cornelissen, A., Cenens, W., Aertsen, A., et al. (2014). Engineered Endolysin-Based “Artilysins” to Combat Multidrug-Resistant Gram-Negative Pathogens. mBio 5. doi:10.1128/mBio.01379-14
Butler, M. J., Bruheim, P., Jovetic, S., Marinelli, F., Postma, P. W., and Bibb, M. J. (2002). Engineering of Primary Carbon Metabolism for Improved Antibiotic Production in Streptomyces Lividans. Appl. Environ. Microbiol. 68, 4731–4739. doi:10.1128/AEM.68.10.4731-4739.2002
Byrne, B., Carmody, M., Gibson, E., Rawlings, B., and Caffrey, P. (2003). Biosynthesis of Deoxyamphotericins and Deoxyamphoteronolides by Engineered Strains of Streptomyces Nodosus. Chem. Biol. 10, 1215–1224. doi:10.1016/j.chembiol.2003.12.001
Cai, D., Zhang, B., Zhu, J., Xu, H., Liu, P., Wang, Z., et al. (2020). Enhanced Bacitracin Production by Systematically Engineering S-Adenosylmethionine Supply Modules in Bacillus Licheniformis. Front. Bioeng. Biotechnol. 8, 305. doi:10.3389/fbioe.2020.00305
Cai, D., Zhu, J., Zhu, S., Lu, Y., Zhang, B., Lu, K., et al. (2019). Metabolic Engineering of Main Transcription Factors in Carbon, Nitrogen, and Phosphorus Metabolisms for Enhanced Production of Bacitracin in Bacillus Licheniformis. ACS Synth. Biol. 8, 866–875. doi:10.1021/acssynbio.9b00005
Carbonell, P., Jervis, A. J., Robinson, C. J., Yan, C., Dunstan, M., Swainston, N., et al. (2018). An Automated Design-Build-Test-Learn Pipeline for Enhanced Microbial Production of Fine Chemicals. Commun. Biol. 1, 66. doi:10.1038/s42003-018-0076-9
Chen, J., Chen, Z., Yuan, K., Huang, Z., and Mao, M. (2021). Recombinant Bacteriophage T4 Rnl1 Impacts Streptococcus Mutans Biofilm Formation. J. Oral Microbiol. 13, 1–8. doi:10.1080/20002297.2020.1860398
Chen, W., Huang, T., He, X., Meng, Q., You, D., Bai, L., et al. (2009). Characterization of the Polyoxin Biosynthetic Gene Cluster from Streptomyces Cacaoi and Engineered Production of Polyoxin H. J. Biol. Chem. 284, 10627–10638. doi:10.1074/jbc.M807534200
Culp, E. J., Waglechner, N., Wang, W., Fiebig-Comyn, A. A., Hsu, Y. P., Koteva, K., et al. (2020). Evolution-Guided Discovery of Antibiotics That Inhibit Peptidoglycan Remodelling. Nature 578 (7796), 582–587.
Dedrick, R. M., Guerrero-Bustamante, C. A., Garlena, R. A., Russell, D. A., Ford, K., Harris, K., et al. (2019). Engineered Bacteriophages for Treatment of a Patient With a Disseminated Drug-Resistant Mycobacterium Abscessus. Nature Med. 25, 730–733. doi:10.1038/s41591-019-0437-z
Edgar, R., Friedman, N., Molshanski-Mor, S., and Qimron, U. (2012). Reversing Bacterial Resistance to Antibiotics by Phage-Mediated Delivery of Dominant Sensitive Genes. Appl. Environ. Microbiol. 78, 744–751. doi:10.1128/AEM.05741-11
El Karoui, M., Hoyos-Flight, M., and Fletcher, L. (2019). Future Trends in Synthetic Biology-A Report. Front. Bioeng. Biotechnol. 7, 175. doi:10.3389/fbioe.2019.00175
Eppelmann, K., Doekel, S., and Marahiel, M. A. (2001). Engineered Biosynthesis of the Peptide Antibiotic Bacitracin in the Surrogate Host Bacillus Subtilis. J. Biol. Chem. 276, 34824–34831. doi:10.1074/jbc.M104456200
Eustáquio, A. S., Gust, B., Li, S.-M., Pelzer, S., Wohlleben, W., Chater, K. F., et al. (2004). Production of 8′-Halogenated and 8′-Unsubstituted Novobiocin Derivatives in Genetically Engineered Streptomyces Coelicolor Strains. Chem. Biol. 11, 1561–1572. doi:10.1016/j.chembiol.2004.09.009
Fu, W., Forster, T., Mayer, O., Curtin, J. J., Lehman, S. M., Donlan, R. M., et al. (2010). Bacteriophage Cocktail for the Prevention of Biofilm Formation by Pseudomonas Aeruginosa on Catheters in an in vitro Model System. Antimicrob. Agents Chemother. 54, 397–404. doi:10.1128/AAC.00669-09
Gupta, S., Bram, E. E., and Weiss, R. (2013). Genetically Programmable Pathogen Sense and Destroy. ACS Synth. Biol. 2, 715–723. doi:10.1021/sb4000417
Han, A. R., Shinde, P. B., Park, J. W., Cho, J., Lee, S. R., Ban, Y. H., et al. (2012). Engineered Biosynthesis of Glycosylated Derivatives of Narbomycin and Evaluation of Their Antibacterial Activities. Appl. Microbiol. Biotechnol. 93, 1147–1156. doi:10.1007/s00253-011-3592-9
Hennig, S., Rödel, G., and Ostermann, K. (2015). Artificial Cell-Cell Communication as an Emerging Tool in Synthetic Biology Applications. J. Biol. Eng. 9, 13–12. doi:10.1186/S13036-015-0011-2/FIGURES/2
Holowko, M. B., Wang, H., Jayaraman, P., and Poh, C. L. (2016). Biosensing Vibrio cholerae with Genetically Engineered Escherichia coli. ACS Synth. Biol. 5, 1275–1283. doi:10.1021/acssynbio.6b00079
Huh, J.-H., Kim, D.-j., Zhao, X.-Q., Li, M., Jo, Y.-Y., Yoon, T.-M., et al. (2004). Widespread Activation of Antibiotic Biosynthesis byS-Adenosylmethionine in Streptomycetes. FEMS Microbiol. Lett. 238, 439–447. doi:10.1111/j.1574-6968.2004.tb09787.x
Hwang, S., Lee, N., Cho, S., Palsson, B., and Cho, B. K. (2020). Repurposing Modular Polyketide Synthases and Non-ribosomal Peptide Synthetases for Novel Chemical Biosynthesis. Front. Mol. Biosci. 7, 87. doi:10.3389/fmolb.2020.00087
Ji, C.-H., Kim, H., Je, H.-W., Kwon, H., Lee, D., and Kang, H.-S. (2022). Top-down Synthetic Biology Approach for Titer Improvement of Clinically Important Antibiotic Daptomycin in Streptomyces Roseosporus. Metab. Eng. 69, 40–49. doi:10.1016/j.ymben.2021.10.013
Kim, H. U., Charusanti, P., Lee, S. Y., and Weber, T. (2016). Metabolic Engineering with Systems Biology Tools to Optimize Production of Prokaryotic Secondary Metabolites. Nat. Prod. Rep. 33, 933–941. doi:10.1039/C6NP00019C
Li, D., Tian, Y., Liu, X., Wang, W., Li, Y., Tan, H., et al. (2021a). Reconstitution of a Mini‐gene Cluster Combined with Ribosome Engineering Led to Effective Enhancement of Salinomycin Production in Streptomyces Albus. Microb. Biotechnol. 14, 2356–2368. doi:10.1111/1751-7915.13686
Li, Y.-P., Bu, Q.-T., Li, J.-F., Xie, H., Su, Y.-T., Du, Y.-L., et al. (2021b). Genome-based Rational Engineering of Actinoplanes Deccanensis for Improving Fidaxomicin Production and Genetic Stability. Bioresour. Technol. 330, 124982. doi:10.1016/j.biortech.2021.124982
Liao, L., Cheng, Y., Liu, S., Zhou, J., An, S., Lv, M., et al. (2014). Production of Novel Antibiotics Zeamines through Optimizing Dickeya Zeae Fermentation Conditions. PLoS One 9, e116047. doi:10.1371/journal.pone.0116047
Lin, T.-Y. Y., Lo, Y.-H. H., Tseng, P.-W. W., Chang, S.-F. F., Lin, Y.-T. T., and Chen, T.-S. S. (2012). A T3 and T7 Recombinant Phage Acquires Efficient Adsorption and a Broader Host Range. PLoS ONE 7, 1–10. doi:10.1371/journal.pone.0030954
Lubkowicz, D., Ho, C. L., Hwang, I. Y., Yew, W. S., Lee, Y. S., and Chang, M. W. (2018). Reprogramming Probiotic Lactobacillus Reuteri as a Biosensor for Staphylococcus aureus Derived AIP-I Detection. ACS Synth. Biol. 7, 1229–1237. doi:10.1021/acssynbio.8b00063
Ma, J., Huang, H., Xie, Y., Liu, Z., Zhao, J., Zhang, C., et al. (2017). Biosynthesis of Ilamycins Featuring Unusual Building Blocks and Engineered Production of Enhanced Anti-tuberculosis Agents. Nat. Commun. 8, 1–10. doi:10.1038/s41467-017-00419-5
Makitrynskyy, R., Tsypik, O., and Bechthold, A. (2021). Genetic Engineering of Streptomyces Ghanaensis ATCC14672 for Improved Production of Moenomycins. Microorganisms 10, 30. doi:10.3390/microorganisms10010030
Martínez-Núñez, M. A., and López, V. E. L. y. (2016). Nonribosomal Peptides Synthetases and Their Applications in Industry. Sustain. Chem. Process 4, 13. doi:10.1186/s40508-016-0057-6
Masschelein, J., Clauwers, C., Awodi, U. R., Stalmans, K., Vermaelen, W., Lescrinier, E., et al. (2015). A Combination of Polyunsaturated Fatty Acid, Nonribosomal Peptide and Polyketide Biosynthetic Machinery Is Used to Assemble the Zeamine Antibiotics. Chem. Sci. 6, 923–929. doi:10.1039/C4SC01927J
Masschelein, J., Mattheus, W., Gao, L.-J., Moons, P., Van Houdt, R., Uytterhoeven, B., et al. (2013). A PKS/NRPS/FAS Hybrid Gene Cluster from Serratia Plymuthica RVH1 Encoding the Biosynthesis of Three Broad Spectrum, Zeamine-Related Antibiotics. PLoS One 8, e54143. doi:10.1371/journal.pone.0054143
McCarty, N. S., and Ledesma-Amaro, R. (2019). Synthetic Biology Tools to Engineer Microbial Communities for Biotechnology. Trends Biotechnol. 37, 181–197. doi:10.1016/j.tibtech.2018.11.002
Meng, S., Wu, H., Wang, L., Zhang, B., and Bai, L. (2017). Enhancement of Antibiotic Productions by Engineered Nitrate Utilization in Actinomycetes. Appl. Microbiol. Biotechnol. 101, 5341–5352. doi:10.1007/s00253-017-8292-7
Miao, V., Coëffet-Le Gal, M.-F., Nguyen, K., Brian, P., Penn, J., Whiting, A., et al. (2006). Genetic Engineering in Streptomyces Roseosporus to Produce Hybrid Lipopeptide Antibiotics. Chem. Biol. 13, 269–276. doi:10.1016/j.chembiol.2005.12.012
Moosmann, D., Mokeev, V., Kulik, A., Osipenkov, N., Kocadinc, S., Ort-Winklbauer, R., et al. (2020). Genetic Engineering Approaches for the Fermentative Production of Phenylglycines. Appl. Microbiol. Biotechnol. 104, 3433–3444. doi:10.1007/s00253-020-10447-9
Morris, M. K., Saez-Rodriguez, J., Sorger, P. K., and Lauffenburger, D. A. (2010). Logic-Based Models for the Analysis of Cell Signaling Networks. Biochem. 49, 3216–3224. doi:10.1021/bi902202q
Nivina, A., Yuet, K. P., Hsu, J., and Khosla, C. (2019). Evolution and Diversity of Assembly-Line Polyketide Synthases. Chem. Rev. 119, 12524–12547. doi:10.1021/acs.chemrev.9b00525
Olano, C., Lombó, F., Méndez, C., and Salas, J. A. (2008). Improving Production of Bioactive Secondary Metabolites in Actinomycetes by Metabolic Engineering. Metab. Eng. 10, 281–292. doi:10.1016/j.ymben.2008.07.001
Opgenorth, P., Costello, Z., Okada, T., Goyal, G., Chen, Y., Gin, J., et al. (2019). Lessons from Two Design-Build-Test-Learn Cycles of Dodecanol Production in Escherichia coli Aided by Machine Learning. ACS Synth. Biol. 8, 1337–1351. doi:10.1021/acssynbio.9b00020
Palazzotto, E., Tong, Y., Lee, S. Y., and Weber, T. (2019). Synthetic Biology and Metabolic Engineering of Actinomycetes for Natural Product Discovery. Biotechnol. Adv. 37, 107366. doi:10.1016/j.biotechadv.2019.03.005
Pei, R., and Lamas-Samanamud, G. R. (2014). Inhibition of Biofilm Formation by T7 Bacteriophages Producing Quorum-quenching Enzymes. Appl. Environ. Microbiol. 80, 5340–5348. doi:10.1128/AEM.01434-14
Perrino, G., Hadjimitsis, A., Ledesma-Amaro, R., and Stan, G.-B. (2021). Control Engineering and Synthetic Biology: Working in Synergy for the Analysis and Control of Microbial Systems. Curr. Opin. Microbiol. 62, 68–75. doi:10.1016/j.mib.2021.05.004
Petzold, C. J., Chan, L. J. G., Nhan, M., and Adams, P. D. (2015). Analytics for Metabolic Engineering. Front. Bioeng. Biotechnol. 3, 135. doi:10.3389/fbioe.2015.00135
Sakai, A., Mitsumori, A., Furukawa, M., Kinoshita, K., Anzai, Y., and Kato, F. (2012). Production of a Hybrid 16-membered Macrolide Antibiotic by Genetic Engineering of Micromonospora Sp. TPMA0041. J. Ind. Microbiol. Biotechnol. 39, 1693–1701. doi:10.1007/s10295-012-1173-2
Schooley, R. T., Biswas, B., Gill, J. J., Hernandez-Morales, A., Lancaster, J., Lessor, L., et al. (2017). Development and Use of Personalized Bacteriophage-Based Therapeutic Cocktails to Treat a Patient with a Disseminated Resistant Acinetobacter Baumannii Infection. Antimicrob. Agents Chemother. 61, e00954–17. doi:10.1128/AAC.00954-17
Sharma, S., Chatterjee, S., Datta, S., Prasad, R., Dubey, D., Prasad, R. K., et al. (2017). Bacteriophages and its Applications: an Overview. Folia Microbiol. 62, 17–55. doi:10.1007/s12223-016-0471-x
Shomar, H., Gontier, S., Van Den Broek, N. J. F., Tejeda Mora, H., Noga, M. J., Hagedoorn, P.-L., et al. (2018). Metabolic Engineering of a Carbapenem Antibiotic Synthesis Pathway in Escherichia coli. Nat. Chem. Biol. 14, 794–800. doi:10.1038/s41589-018-0084-6
Song, K., Wei, L., Liu, J., Wang, J., Qi, H., and Wen, J. (2017). Engineering of the LysR Family Transcriptional Regulator FkbR1 and its Target Gene to Improve Ascomycin Production. Appl. Microbiol. Biotechnol. 101, 4581–4592. doi:10.1007/S00253-017-8242-4
Ventola, C. L. (2015). The Antibiotic Resistance Crisis: Part 1: Causes and Threats. Pharm. Ther. 40 (4), 277.
Wang, B., Kitney, R. I., Joly, N., and Buck, M. (2011). Engineering Modular and Orthogonal Genetic Logic Gates for Robust Digital-Like Synthetic Biology. Nat. Commun. 2, 508. doi:10.1038/ncomms1516
Wang, K., and Nicholaou, M. (2017). Suppression of Antimicrobial Resistance in MRSA Using CRISPR-dCas9.. Am. Soc. Clin. Lab. Sci. 30, 207–213. doi:10.29074/ascls.30.4.207
Wang, W.-F., Xiao, H., and Zhong, J.-J. (2021). Biosynthesis of a Novel Ganoderic Acid by Expressing CYP Genes from Ganoderma Lucidum in Saccharomyces cerevisiae. Appl. Microbiol. Biotechnol. 106, 523–534. doi:10.1007/s00253-021-11717-w
Wang, Y., Boghigian, B. A., and Pfeifer, B. A. (2007). Improving Heterologous Polyketide Production in Escherichia coli by Overexpression of an S-Adenosylmethionine Synthetase Gene. Appl. Microbiol. Biotechnol. 77, 367–373. doi:10.1007/s00253-007-1172-9
Whitford, C. M., Cruz-Morales, P., Keasling, J. D., and Weber, T. (2021). The Design-Build-Test-Learn Cycle for Metabolic Engineering of Streptomycetes. Essays Biochem. 65, 261–275. doi:10.1042/EBC20200132
World Health Organization, [WHO] (2017). Who Publishes List of Bacteria for Which new Antibiotics are Urgently Needed. Saudi Med. J.Available at: https://www.who.int/news-room/detail/27-02-2017-who-publishes-list-of-bacteria-for-which-new-antibiotics-are-urgently-needed.
Wu, J., Zhang, H.-B., Xu, J.-L., Cox, R. J., Simpson, T. J., and Zhang, L.-H. (2010). 13CLabeling Reveals Multiple Amination Reactions in the Biosynthesis of a Novel Polyketide Polyamine Antibioticzeamine from Dickeya Zeae. Chem. Commun. 46, 333–335. doi:10.1039/B916307G
Wu, M.-C., Struck, A.-W., Nunns, L., Styles, M. Q., Micklefield, J., and Law, B. J. C. (2015). Engineered Biosynthesis of Enduracidin Lipoglycopeptide Antibiotics Using the Ramoplanin Mannosyltransferase Ram29. Microbiol. (United Kingdom) 161, 1338–1347. doi:10.1099/mic.0.000095
Wu, Y., Wang, C.-W., Wang, D., and Wei, N. (2021). A Whole-Cell Biosensor for Point-of-Care Detection of Waterborne Bacterial Pathogens. ACS Synth. Biol. 10, 333–344. doi:10.1021/acssynbio.0c00491
Xu, M., Wang, W., Waglechner, N., Culp, E. J., Guitor, A. K., and Wright, G. D. (2020). GPAHex-A Synthetic Biology Platform for Type IV-V Glycopeptide Antibiotic Production and Discovery. Nat. Commun. 11, 5232. doi:10.1038/s41467-020-19138-5
Yacoby, I., Bar, H., and Benhar, I. (2007). Targeted Drug-Carrying Bacteriophages as Antibacterial Nanomedicines. Antimicrob. Agents Chemother. 51, 2156–2163. doi:10.1128/AAC.00163-07
Ye, C., Ng, I.-S., Jing, K., and Lu, Y. (2014). Direct Proteomic Mapping of Streptomyces Roseosporus NRRL 11379 with Precursor and Insights into Daptomycin Biosynthesis. J. Biosci. Bioeng. 117, 591–597. doi:10.1016/j.jbiosc.2013.10.021
Yehl, K., Lemire, S., Yang, A. C., Ando, H., Mimee, M., Torres, M. D. T., et al. (2019). Engineering Phage Host-Range and Suppressing Bacterial Resistance through Phage Tail Fiber Mutagenesis. Cell 179, 459–469.e9. doi:10.1016/j.cell.2019.09.015
Yim, G., Thaker, M. N., Koteva, K., and Wright, G. (2014). Glycopeptide Antibiotic Biosynthesis. J. Antibiot. 67, 31–41. doi:10.1038/ja.2013.117
Yim, G., Wang, W., Thaker, M. N., Tan, S., and Wright, G. D. (2016). How to Make a Glycopeptide: A Synthetic Biology Approach to Expand Antibiotic Chemical Diversity. ACS Infect. Dis. 2, 642–650. doi:10.1021/acsinfecdis.6b00105
Zhang, F., Gao, D., Lin, J., Zhu, M., Zhuang, Z., Duan, Y., et al. (2020). Construction of Inducible Genetic Switch for the Global Regulator WblA to Sustain Both Overproduction of Tiancimycins and On-Demand Sporulation in Streptomyces Sp. CB03234. ACS Synth. Biol. 9, 1460–1467. doi:10.1021/acssynbio.0c00114
Zhu, Q., Li, J., Ma, J., Luo, M., Wang, B., Huang, H., et al. (2012). Discovery and Engineered Overproduction of Antimicrobial Nucleoside Antibiotic A201A from the Deep-Sea Marine Actinomycete Marinactinospora Thermotolerans SCSIO 00652. Antimicrob. Agents Chemother. 56, 110–114. doi:10.1128/AAC.05278-11
Keywords: synthetic biology, antimicrobial resistance, genetic circuits, antibiotics, phages, whole-cell engineering
Citation: León-Buitimea A, Balderas-Cisneros FdJ, Garza-Cárdenas CR, Garza-Cervantes JA and Morones-Ramírez JR (2022) Synthetic Biology Tools for Engineering Microbial Cells to Fight Superbugs. Front. Bioeng. Biotechnol. 10:869206. doi: 10.3389/fbioe.2022.869206
Received: 04 February 2022; Accepted: 18 April 2022;
Published: 04 May 2022.
Edited by:
Jean Marie François, Institut Biotechnologique de Toulouse (INSA), FranceReviewed by:
Ho Chun Loong, Southern University of Science and Technology, ChinaJingyu Chen, China Agricultural University, China
Copyright © 2022 León-Buitimea, Balderas-Cisneros, Garza-Cárdenas, Garza-Cervantes and Morones-Ramírez. This is an open-access article distributed under the terms of the Creative Commons Attribution License (CC BY). The use, distribution or reproduction in other forums is permitted, provided the original author(s) and the copyright owner(s) are credited and that the original publication in this journal is cited, in accordance with accepted academic practice. No use, distribution or reproduction is permitted which does not comply with these terms.
*Correspondence: José Rubén Morones-Ramírez, jose.moronesrmr@uanl.edu.mx